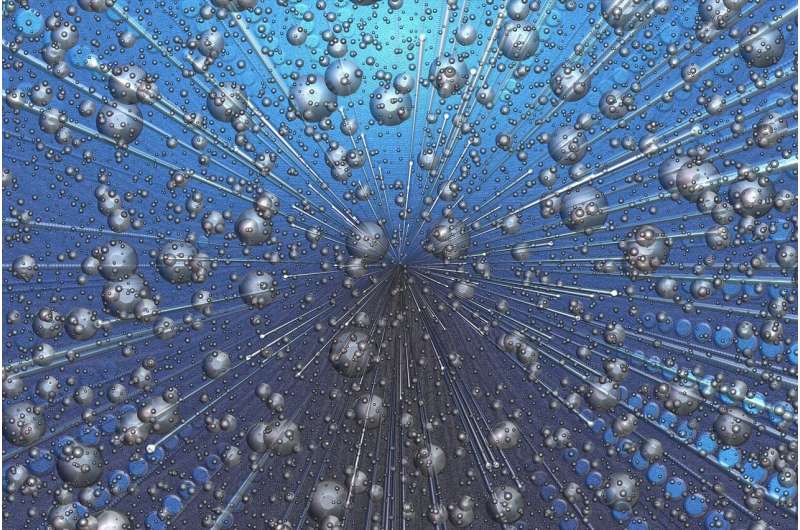
Say you notice a sudden drop in temperature on both your patio and kitchen thermometers. At first, you think it's because of a cold snap, so you crank up the heat in your home. Then you realize that while the outside has indeed become colder, inside, someone left the refrigerator door open.
Why it matters
Quantum sensors harness the quantum properties of atoms or atom-like systems to pick up tiny signals—such as the magnetic fields arising from the motion of single electrons. These fields are 100,000 times weaker than that of a refrigerator magnet. Only ultrasensitive tools such as quantum sensors can make measurements at nature's smallest scales.
Quantum sensors are expected to be powerful. NV centers, for example, can distinguish features separated by a mere one ten-thousandth of the width of a human hair. With that kind of hyperzoom capability, NV centers could be placed in living cells for an inside, up-close look at how they function. Scientists could even use them to pinpoint the causes of disease.
"What make NVs special is their spatial resolution," Kolkowitz said. "That's useful for imaging the magnetic fields from an exotic material or seeing the structure of individual proteins."
With the Kolkowitz team's new method for sensing magnetic field strengths at multiple points simultaneously, scientists could one day be able to map atom-level changes in magnetism through time and space.
How it works
How did the team make these informative measurements? They got granular.
Rather than average over many raw values to arrive at the overall magnetic field strength, the researchers kept track of individual readings at each NV center, and then applied a mathematical maneuver called "covariance" to the two lists.
Comparing the covariance-calculated figures—which capture more detail than a couple of raw averages—let them see whether the fields were correlated.
"We're doing that averaging differently than what's been done in the past, so we don't lose this information in the process of averaging," Kolkowitz said "That's part of what's special here."
So why hasn't covariance magnetometry, as the method is called, been tested before now?
For one, the team had to build an experimental setup for taking simultaneous measurements at multiple NV centers. This microscope was built by the team at Princeton, led by Professor Nathalie de Leon, a member of the Co-Design Center for Quantum Advantage, another DOE National Quantum Information Science Research Center, led by Brookhaven National Laboratory.
For another, covariance magnetometry works only when the individual measurements of these tiny magnetic fields are highly reliable. (A readout is only as good as its contributing measurements.) That's why the researchers used a special technique called spin-to-charge conversion, which produces a raw reading with more information about the magnetic field for each measurement than other commonly used tools.
With spin-to-charge conversion, individual measurements take longer. That's the price scientists pay for higher reliability.
However, when combined with covariance to measure minuscule, correlated magnetic fields, it saves buckets of time.
"Using the conventional method, you'd have to average for 10 full days continuously to get one piece of data to say that you saw this correlated nanotesla signal," Kolkowitz said, "whereas with this new method, it's an hour or two."
By integrating covariance information with spin-to-charge conversion, researchers can gain access to atomic and subatomic details they didn't have before, supercharging the already powerful capabilities of quantum sensing.
"As far as I know, this is something people hadn't tried to do, and that's why we see these correlations where nobody else was able to," Kolkowitz said. "You really win from that."
More information: Jared Rovny et al, Nanoscale covariance magnetometry with diamond quantum sensors, Science (2022). DOI: 10.1126/science.ade9858
Original Text (This is the original text for your reference.)
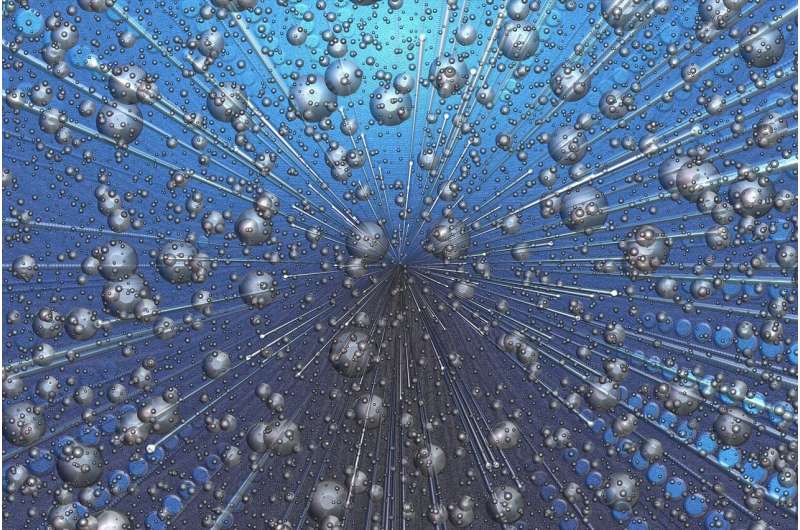
Say you notice a sudden drop in temperature on both your patio and kitchen thermometers. At first, you think it's because of a cold snap, so you crank up the heat in your home. Then you realize that while the outside has indeed become colder, inside, someone left the refrigerator door open.
Why it matters
Quantum sensors harness the quantum properties of atoms or atom-like systems to pick up tiny signals—such as the magnetic fields arising from the motion of single electrons. These fields are 100,000 times weaker than that of a refrigerator magnet. Only ultrasensitive tools such as quantum sensors can make measurements at nature's smallest scales.
Quantum sensors are expected to be powerful. NV centers, for example, can distinguish features separated by a mere one ten-thousandth of the width of a human hair. With that kind of hyperzoom capability, NV centers could be placed in living cells for an inside, up-close look at how they function. Scientists could even use them to pinpoint the causes of disease.
"What make NVs special is their spatial resolution," Kolkowitz said. "That's useful for imaging the magnetic fields from an exotic material or seeing the structure of individual proteins."
With the Kolkowitz team's new method for sensing magnetic field strengths at multiple points simultaneously, scientists could one day be able to map atom-level changes in magnetism through time and space.
How it works
How did the team make these informative measurements? They got granular.
Rather than average over many raw values to arrive at the overall magnetic field strength, the researchers kept track of individual readings at each NV center, and then applied a mathematical maneuver called "covariance" to the two lists.
Comparing the covariance-calculated figures—which capture more detail than a couple of raw averages—let them see whether the fields were correlated.
"We're doing that averaging differently than what's been done in the past, so we don't lose this information in the process of averaging," Kolkowitz said "That's part of what's special here."
So why hasn't covariance magnetometry, as the method is called, been tested before now?
For one, the team had to build an experimental setup for taking simultaneous measurements at multiple NV centers. This microscope was built by the team at Princeton, led by Professor Nathalie de Leon, a member of the Co-Design Center for Quantum Advantage, another DOE National Quantum Information Science Research Center, led by Brookhaven National Laboratory.
For another, covariance magnetometry works only when the individual measurements of these tiny magnetic fields are highly reliable. (A readout is only as good as its contributing measurements.) That's why the researchers used a special technique called spin-to-charge conversion, which produces a raw reading with more information about the magnetic field for each measurement than other commonly used tools.
With spin-to-charge conversion, individual measurements take longer. That's the price scientists pay for higher reliability.
However, when combined with covariance to measure minuscule, correlated magnetic fields, it saves buckets of time.
"Using the conventional method, you'd have to average for 10 full days continuously to get one piece of data to say that you saw this correlated nanotesla signal," Kolkowitz said, "whereas with this new method, it's an hour or two."
By integrating covariance information with spin-to-charge conversion, researchers can gain access to atomic and subatomic details they didn't have before, supercharging the already powerful capabilities of quantum sensing.
"As far as I know, this is something people hadn't tried to do, and that's why we see these correlations where nobody else was able to," Kolkowitz said. "You really win from that."
More information: Jared Rovny et al, Nanoscale covariance magnetometry with diamond quantum sensors, Science (2022). DOI: 10.1126/science.ade9858
Comments
Something to say?
Log in or Sign up for free